Applying the Goldilocks Principle to DNA Structure
Scientists consider the form that may be “just right” for scripting gene expression
Published Date
By:
- Cynthia Dillon
Share This:
Article Content
The Goldilocks of fairy-tale fame knew something about porridge. It needed to be just right—neither too hot nor too cold. Same with furniture—neither too hard nor too soft. In a different context, scientists at UC San Diego know something about DNA. They know that the strands of our genetic code, if extended, would measure two meters, or about six feet. They also know that the strands fold into and move within the cell nucleus the size of about a hundredth of a millimeter. But they don’t know how and in what state of matter this occurs, so they decided to check.
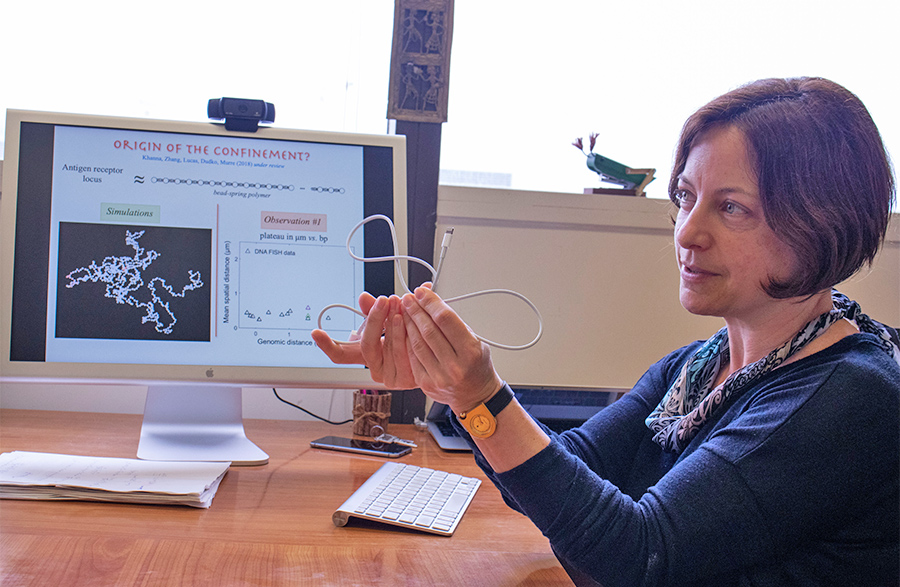
Olga Dudko uses a charge cord to demonstrate genomic interactions within the nucleus of a cell. Photo by Michelle Fredricks, UC San Diego Physical Sciences
Inspired by ideas from the physics of phase transitions and polymer physics, researchers in the Divisions of Physical and Biological Sciences at UC San Diego set out specifically to determine the organization of DNA inside the nucleus of a living cell. The findings of their study, recently published in Nature Communications, suggest that the phase state of the genomic DNA is “just right”—a gel poised at the phase boundary between gel and sol, the solid-liquid phase transition.
Think of pudding, panna cotta—or even porridge. The consistency of these delectables must be just right to be ideally enjoyed. Just as the “sol-gel” phase transition, according to the scientists, seems just right for explaining the timing of genomic interactions that dictate gene expression and somatic recombination.
“This finding points to a general physical principle of chromosomal organization, which has important implications for many key processes in biology, from antibody production to tissue differentiation,” said Olga Dudko, a theoretical biophysicist and professor in the Department of Physics at UC San Diego, who collaborated with colleague Cornelis Murre, a distinguished professor in the Section of Molecular Biology, on the study.
Along with Dudko’s former graduate student Yaojun Zhang, now a postdoctoral researcher at Princeton, and Murre’s postdoctoral scholar Nimish Khanna, the team collected and analyzed data on DNA motion inside live mammalian B-cells from mice to understand how remote genomic interactions generate a diverse pool of antibodies by the adaptive immune system.
In mammals, such as rodents and humans, immunoglobin gene segments are arranged in groups of variable (V), diversity (D) and joining (J) segments. These V, D and J segments randomly combine through the process of somatic recombination. This occurs before antigen contact and during B-cell development in the immune system’s lymphoid tissue, or bone marrow. These random genetic interactions result in diverse protein codes that match up with antigens which activate lymphocytes.
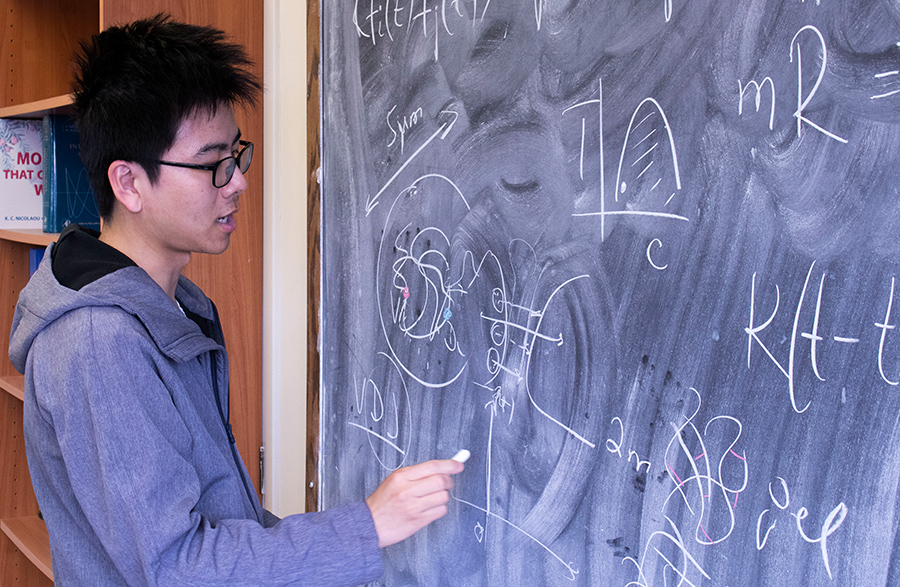
Physics graduate student Bin Wang, who works with Dudko, charts temporal and spatial aspects of the research. Photo by Michelle Fredricks, UC San Diego Physical Sciences
The scientists examined the various interactions between V and DJ gene segments. While how exactly these interactions occur remains unknown, the UC San Diego researchers developed a strategy to track V and DJ motion in B-lymphocytes. They found that V and DJ segments were trapped in configurations that allowed local motion only—in other words, the segments remained spatially proximal if they were initially close or they remained separate if they were initially spatially distant. The researchers also observed, within a subset of cells, abrupt changes in V and DJ motion, plausibly caused by temporal changes in chromatin.
By comparing experimental and simulated data, the scientists concluded that constrained motion is imposed by a network of cross-linked chromatin chains, or a mesh of bridges between the DNA strands that are characteristic of a gel phase. Yet, the amount of these cross-links is “just right” to poise the DNA near the sol phase—a liquid phase describing a solution of uncross-linked chains.
This pattern suggested to the scientists that a certain organizational principle of genomic DNA exists—proximity to the sol-gel phase transition—which explains how the genome can simultaneously possess stability and responsiveness within the nucleus.
These results indicate that the packing pattern of DNA within a cell’s nucleus has consequences for a cell’s fate—whether it becomes a live or diseased cell.
“We have rigorous theories from physics—abstract principles and mathematical equations. We have state-of-the-art experiments on biology—innovative tracking of gene segments in live mammalian cell nuclei,” noted Zhang. “It really amazes and excites me when the two aspects merge coherently into one story, where physics is not just a tool to describe the dynamics of gene segments, but helps to pinpoint the physical state of the genome, and further sheds light on the impact of the physical properties of this state on its biological function.”
This study was supported by grants from the National Institutes of Health (U54DK107977, U54DK24230, AI082850, AI00880 and AI09599); the Princeton Center for Theoretical Science, the National Science Foundation (grant PHY1607612); and the NSF Center for the Physics of Biological Function (PHY1734030). Imaging was performed at the University of California San Diego School of Medicine (Core Grant P30 NSO47101).
Share This:
You May Also Like
Stay in the Know
Keep up with all the latest from UC San Diego. Subscribe to the newsletter today.