Diamonds, Pencils Inspire Scientists to Create Multipurpose Protein Tool
Researchers’ protein crystals generate surprising electrical charge useful for various materials
Published Date
By:
- Cynthia Dillon
Share This:
Article Content
Diamonds and pencil lead—one hard, the other soft enough to write with—share something in common. They are both made from carbon atoms. But the way the atoms bond is what accounts for their different textures. This got a team of researchers from UC San Diego, Pacific Northwest National Laboratory (PNNL) and the University of Washington thinking—relative to artificial protein design. How can proteins be used in the same way to form different materials with unique properties from a single building block?
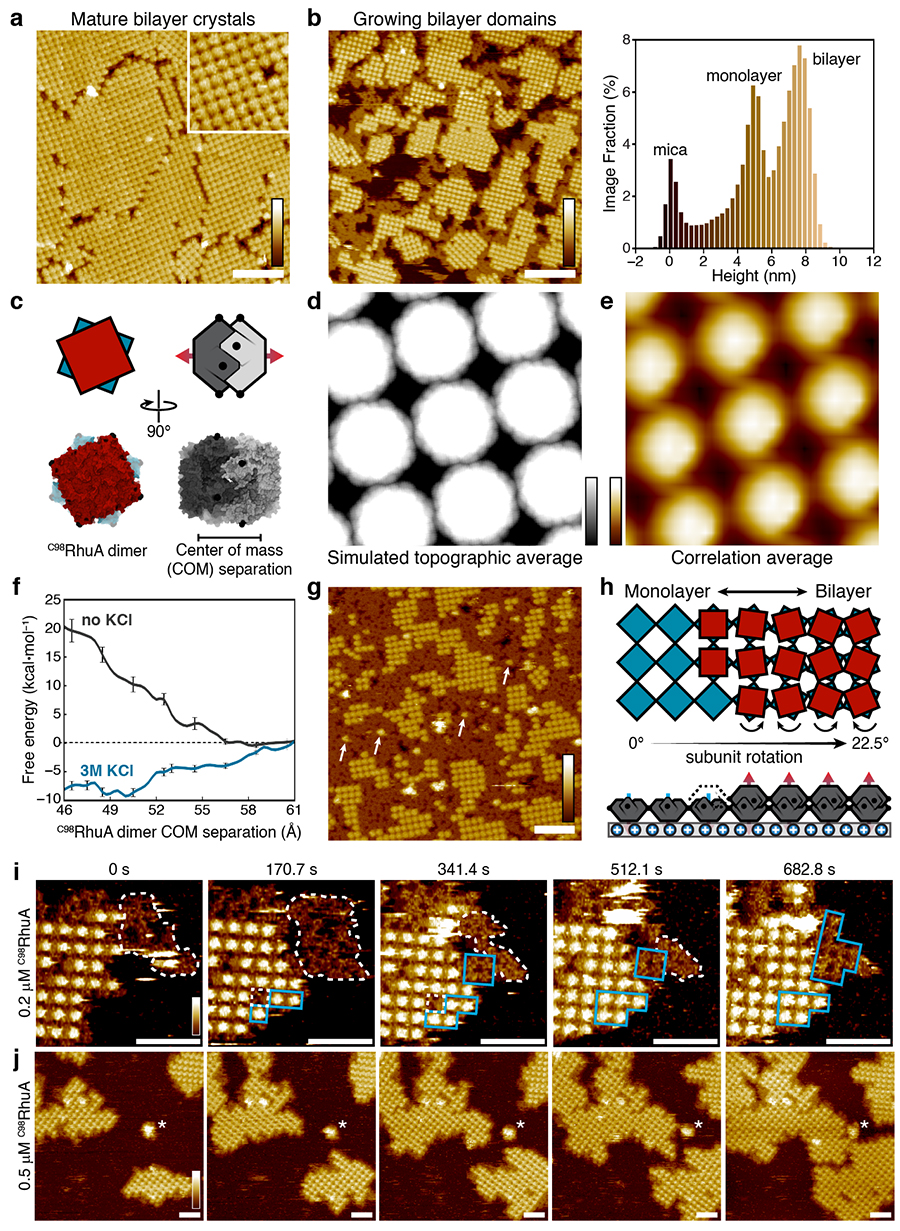
Surface self-assembly of bilayer C98RhuA crystals. High-resolution AFM images of a) fully formed and b) growing bilayer crystals, with image height value histogram shown on right. c) C98RhuA dimer structure. d) Simulated and e) experimental average AFM images for the bilayer structure. f) Calculated energies of C98RhuA dimerization in the presence/absence of 3M KCl, showing that KCl favors the formation of dimers. g) Self-assembly of very high concentrations of C98RhuA, resulting in disordered monolayers which cannot template the growth of a second layer, as indicated by the rarity of isolated 2nd layer monomers (arrows) outside of crystalline domains. h) Proposed self-templated self-assembly pathway for bilayer crystals. i) At lower protein concentrations, two growth mechanisms could be observed: the underlying monolayers grow via “nonclassical” amorphous (white contour)-to-crystalline (blue regions) transitions at edges, which subsequently template second layer growth through “classical” (monomer-by-monomer) addition. j) At higher protein concentrations, crystals appeared only as bilayers, indicating that second-layer formation is very fast and limited by the growth rate of the first layer. Scale bars (white): 100 nm (a/b/g), 50 nm (i/j). Height ranges (gradient): 10 nm (a/b/j), 2 nm (d), 4 nm (e), 12 nm (g), 8 nm (i). Images courtesy of Rob Alberstein
There has been a lot of scientific effort to understand design principles for creating purpose-built proteins for all types of technological applications, including medicine, chemical catalysis and stimuli-responsive protein assemblies. The latest findings from this group of researchers, published in Nature Communications, demonstrate that it is possible to create a protein “multitool” that can be arranged into new and useful ways, depending on its environment. The research provides a deeper understanding of how society can develop new materials with their own unique properties to solve real-world problems that may require specialized solutions.
“Many proteins in our cells already have this ability to serve multiple roles in different contexts, but, so far, engineered, or ‘designed,’ proteins typically can only achieve one type of structure or function,” explained Rob Alberstein, a 2020 Ph.D. alumnus of chemistry and biochemistry at UC San Diego. “This is why we spent a tremendous amount of effort to identify and quantify the molecular forces which create the structures we observed experimentally. Generalizing this type of thinking to the field of artificial protein design is an important step towards achieving behavioral complexity that approaches what is seen in the evolved proteins which exist in our cells today.”
Alberstein, who works with Professor of Chemistry and Biochemistry Akif Tezcan at UC San Diego, explained that when grown on a template, the team’s protein crystals are essentially porous 2D membranes that possess an electric field running across their thickness. This makes these materials, basically, electric magnets or “electrets.” Being porous and asymmetrically charged, purely by nature of how the proteins are connected to each other, charged ions separate across the membrane even without any energy input—like charging a battery. These “electrets” can therefore be imagined to serve roles in water purification, to separate chemical species, or as nanoscale electrical generators.
“Perhaps the most relevant application for our current world, ‘electrets’ are important components in N95 masks, which we use to keep us safe from the current coronavirus pandemic,” said Alberstein. “We may not see proteins in N95 masks anytime soon, but this work opens the possibility for their use in new applications in the future.”
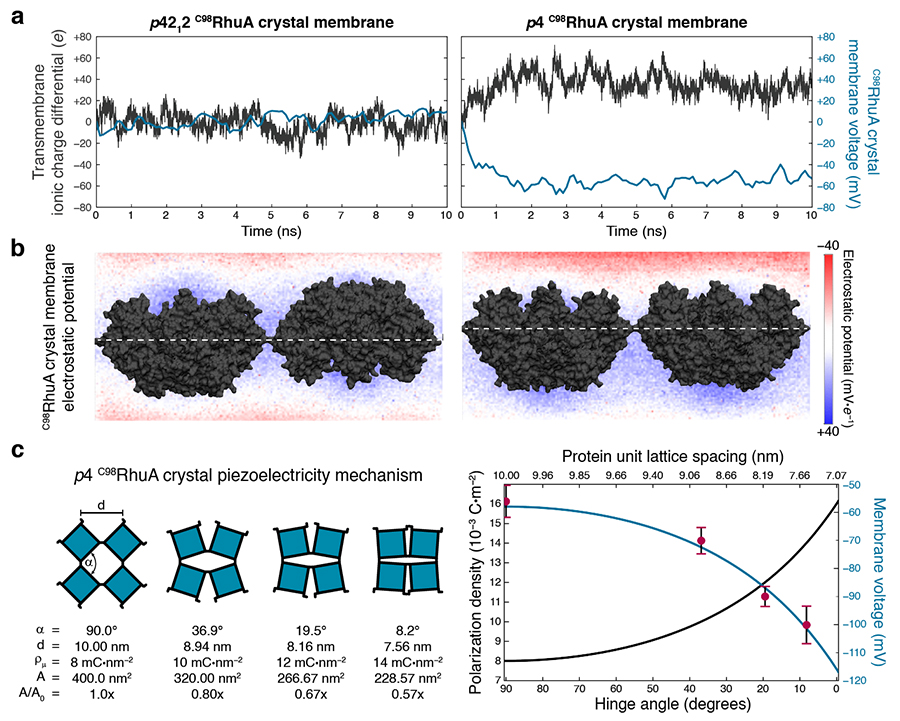
p4 C98RhuA crystals are electrets and piezoelectric materials. a) Simulations quantify the net charge imbalance (due to ion conduction) and resulting electric potential difference across open-state crystals. The dipoles of antiparallel p4212 crystals cancel out and thus induce negligible charge separation, while parallel p4 crystals generate a steady-state 50–60 mV potential difference across the lattice (membrane voltage). b) Calculated electrostatic potential across the central plane of each system directly illustrates the polarization: only p4-symmetry crystals have clearly defined positive and negative poles. c) Piezoelectric properties of p4-symmetry C98RhuA crystals, arising from mechanical coupling between the protein dipole (polarization) density and lattice conformation (left). The magnitude of this effect was predicted analytically for all lattice conformations (blue line, right), which is in excellent agreement with the numerically determined voltages from atomically detailed simulations at discrete conformations (red points, right). Images courtesy of Rob Alberstein
According to the scientists, the crystals they work with generate about 60 to 100 millivolts of charge, and they are mechanically switchable piezoelectric materials (those that can generate an electric charge in response to applied mechanical stress), which are already used in a wide variety of products, including microphones, printers, scientific instruments, push-start ignition devices, clocks and electrical instruments, just to name a few.
Alberstein said the most surprising result of this research was the discovery of the team’s protein’s “unusually strong dipole moment.”
“Due to the protein having positively charged and negatively charged regions on opposite sides of its structure—thus having two poles—it creates an electric field around it, similar to the North/South poles of a bar magnet. Similar to these magnets, the electrical forces which arise from this field causes our proteins to pack antiparallel to one another—just like when you stick two bar magnets together,” explained Alberstein.
According to Alberstein, Tezcan prompted the research colleagues to think about why their solution-grown crystals adopt this particular arrangement.
“The Eureka moment came from my chance reading of a mostly unrelated scientific paper, which made a similar argument for materials constructed from small molecules,” explained Alberstein. “Consequently, we believe this is the first time that protein dipole moments have been strongly implicated as an important consideration for building larger protein structures/materials.”
He said that, having discovered this, he dived down a rabbit hole of literature on electrical materials and learned that parallel-aligned dipoles—like the crystals on the team’s template—have a bulk-scale electric field to match, making them the electrets, as described above, and a material that should have coupled electrical/mechanical properties.
“To then have that prediction borne out by both simulations and purely mathematical formalisms was super exciting and definitely the most unexpected outcome,” said Alberstein. “It was the gift that kept on giving.”
According to Tezcan, the project was a great demonstration of the benefits of combining experiments with theory and computation, which was done in collaboration with Jim De Yoreo and Shuai Zhang at PNNL, who along with the Tezcan Group are a part of the Center of the Science of Synthesis across Scales, a Department of Energy Frontiers Research Center.
“It also highlights the creativity and broad-thinking of UC San Diego graduate students. Having a student like Rob, who introduced our laboratory to computation and immensely expanded our horizons, and working with the De Yoreo Group was an absolute joy.”
This research was supported by the U.S. Department of Energy (DOE), Office of Science, Office of Basic Energy Sciences (BES), as part of the Energy Frontier Research Centers program: SAS–The Center for the Science of Synthesis Across Scales (award no. DE-SC0019288) and the DOE-BES, Division of Materials Sciences, Biomolecular Materials (award no. DE-SC0003844); the Laboratory Directed Research and Development Office through the Materials Synthesis and Simulations Across Scales Initiative; the National Science Foundation, Division of Materials Research (grant no. DMR-1602537); a UC San Diego Distinguished Graduate Student Fellowship and the PNNL, a multi-program national laboratory operated for the DOE by Battelle (contract no. DE-AC05-76RL01830).
Share This:
Stay in the Know
Keep up with all the latest from UC San Diego. Subscribe to the newsletter today.